Clusters of Galaxies
A rich cluster is a complex, multi-component system with hundreds of galaxies, a hot intracluster medium, and dark matter evolving in tightly coupled ways. The properties of clusters are functions both of the processes operating within them and of the underlying cosmology. The determination of cluster properties and their evolution provides fundamental insights into the formation of large scale structure in the universe. Several features make clusters ideal for testing cosmological models. First, clusters are very luminous and are observed both optically and in X-rays to significant distances z ~ 1). Second, since clusters have dynamical timescales which are a significant fraction of the age of the Universe, we can watch them evolve over even modest redshifts. Also since dynamical timescales for clusters are long, the imprint of the initial conditions has not yet been completely erased. Third, since clusters are massive and relatively rare objects, they form only from fairly high peaks in the underlying density field, in the standard scenario. Thus, the cluster mass spectrum is sensitive to the normalization and slope of the power spectrum on cluster scales and is one of the strongest tests of cosmological models. The masses and scales of clusters are such that they comprise a fair sample of the mass of the Universe with representative fractions of the different components. Finally, since cluster formation by gravitational collapse is fairly well understood, comparisons of cluster properties with theoretical predictions for different cosmological models can be used to distinguish among these models.As described below, with ASCA observations, the important first steps have been taken to understand the structure and distribution of dark matter in present epoch rich clusters, the effects of subcluster mergers on the temperature structure of the gas, the source and likely epoch of the heavy element enrichment of the intracluster medium, and finally how clusters evolve over cosmological times in terms of both their gas temperatures and the heavy element enrichment. As also described, the capabilities of ASCA will allow the cosmological parameters of H_0, q_0, and Omega to be determined in the future as samples of distant clusters are observed.
X-ray imaging spectroscopy, as carried out with ASCA, brings a powerful tool to cluster mass measurements (Bahcall & Sarazin 1977 ApJ 213, L99; Mathews 1978 ApJ 218, 413). The X-ray emitting gas, while itself the major contributor to the luminous mass in clusters, also traces, through the hydrostatic equilibrium equation, the total underlying mass. Since the sound crossing time is short compared to the dynamical timescale for most X-ray luminous clusters, the hydrostatic condition appears applicable over the central few Mpc. While the total mass distributions in cool clusters and groups could be measured with ROSAT (e.g. Ponman & Bertram 1993 Nature 363, 51; David et al. 1994 ApJ 428, 544), the broad energy range of ASCA was required to map the gas temperature in the X-ray luminous, massive clusters. The intracluster medium often shows a central cooler region (e.g. Fabian et al. 1994 PASJ 436, L63), surrounded by an isothermal gas region (e.g. Matsuzawa et al. 1996 Waseda meeting; Markevitch and Vikhlinin 1996 ApJ in press), with lower temperatures sometimes found in the outermost regions (e.g. A2163 - Markevitch et al. 1996 ApJ 456, 437). These accurate determinations of the cluster mass allow the gas mass fraction to be measured and the so-called ``baryon catastrophe'' (the high ratio of gas to total mass) to be examined. ASCA studies are now underway to use observations of a flux-limited sample of clusters to determine the present epoch mass function (Bregman and collaborators).
Although it has long been recognized from both X-ray and optical studies that substructure is common in rich clusters (e.g. Dressler & Shectman 1988 AJ 95, 985; Geller & Beers 1982 PASP 94, 421; Jones & Forman 1992 "Clusters and Superclusters" NATO ASI; Bird 1994 AJ 107, 1637, Mohr et al. 1995 ApJ 447, 8), only with ASCA have we been able to clearly observe the effects of the subcluster mergers on the gas temperature structure within clusters. Following a subcluster merger, shock waves will thermalize and equilibrate the gas within a sound crossing time (~ 2 x 10^9 years), allowing deviations from hydrostatic equilibrium to be observed during this time interval. Recent ASCA observations show non-isothermal temperature structures resulting from mergers. While the map of the X-ray emission from the cluster A754 in Figure 6 shows the asymmetrical nature of this system and hints that a subcluster merger may have occured, the ASCA two-dimensional temperature map still retains the dynamical history of that merger (Henriksen and Markevitch 1996 ApJ in press). The temperature distribution derived from ASCA A754 images is very non-uniform with a significantly hotter ridge found along the cluster axis (Figure 6). The ASCA temperature map for A1367 (Churazov et al. 1995 Wurzburg meeting) shows that the smaller subcluster has the higher temperature, due perhaps to shock heating as the smaller subcluster passed through the larger, cooler subcluster. As these examples show, maps of the cluster temperature provide fundamental information on the dynamical history of these systems which cannot be obtained in any other way.
As described below, with ASCA observations, the important first steps have been taken to understand the structure and distribution of dark matter in present epoch rich clusters, the effects of subcluster mergers on the temperature structure of the gas, the source and likely epoch of the heavy element enrichment of the intracluster medium, and finally how clusters evolve over cosmological times in terms of both their gas temperatures and the heavy element enrichment. As also described, the capabilities of ASCA will allow the cosmological parameters of H_0, q_0, and Omega to be determined in the future as samples of distant clusters are observed.
X-ray imaging spectroscopy, as carried out with ASCA, brings a powerful tool to cluster mass measurements (Bahcall & Sarazin 1977 ApJ 213, L99; Mathews 1978 ApJ 218, 413). The X-ray emitting gas, while itself the major contributor to the luminous mass in clusters, also traces, through the hydrostatic equilibrium equation, the total underlying mass. Since the sound crossing time is short compared to the dynamical timescale for most X-ray luminous clusters, the hydrostatic condition appears applicable over the central few Mpc. While the total mass distributions in cool clusters and groups could be measured with ROSAT (e.g. Ponman & Bertram 1993 Nature 363, 51; David et al. 1994 ApJ 428, 544), the broad energy range of ASCA was required to map the gas temperature in the X-ray luminous, massive clusters. The intracluster medium often shows a central cooler region (e.g. Fabian et al. 1994 PASJ 436, L63), surrounded by an isothermal gas region (e.g. Matsuzawa et al. 1996 Waseda meeting; Markevitch and Vikhlinin 1996 ApJ in press), with lower temperatures sometimes found in the outermost regions (e.g. A2163 - Markevitch et al. 1996 ApJ 456, 437). These accurate determinations of the cluster mass allow the gas mass fraction to be measured and the so-called ``baryon catastrophe'' (the high ratio of gas to total mass) to be examined. ASCA studies are now underway to use observations of a flux-limited sample of clusters to determine the present epoch mass function (Bregman and collaborators).
Although it has long been recognized from both X-ray and optical studies that substructure is common in rich clusters (e.g. Dressler & Shectman 1988 AJ 95, 985; Geller & Beers 1982 PASP 94, 421; Jones & Forman 1992 "Clusters and Superclusters" NATO ASI; Bird 1994 AJ 107, 1637, Mohr et al. 1995 ApJ 447, 8), only with ASCA have we been able to clearly observe the effects of the subcluster mergers on the gas temperature structure within clusters. Following a subcluster merger, shock waves will thermalize and equilibrate the gas within a sound crossing time (~ 2 x 10^9 years), allowing deviations from hydrostatic equilibrium to be observed during this time interval. Recent ASCA observations show non-isothermal temperature structures resulting from mergers. While the map of the X-ray emission from the cluster A754 in Figure 6 shows the asymmetrical nature of this system and hints that a subcluster merger may have occured, the ASCA two-dimensional temperature map still retains the dynamical history of that merger (Henriksen and Markevitch 1996 ApJ in press). The temperature distribution derived from ASCA A754 images is very non-uniform with a significantly hotter ridge found along the cluster axis (Figure 6). The ASCA temperature map for A1367 (Churazov et al. 1995 Wurzburg meeting) shows that the smaller subcluster has the higher temperature, due perhaps to shock heating as the smaller subcluster passed through the larger, cooler subcluster. As these examples show, maps of the cluster temperature provide fundamental information on the dynamical history of these systems which cannot be obtained in any other way.
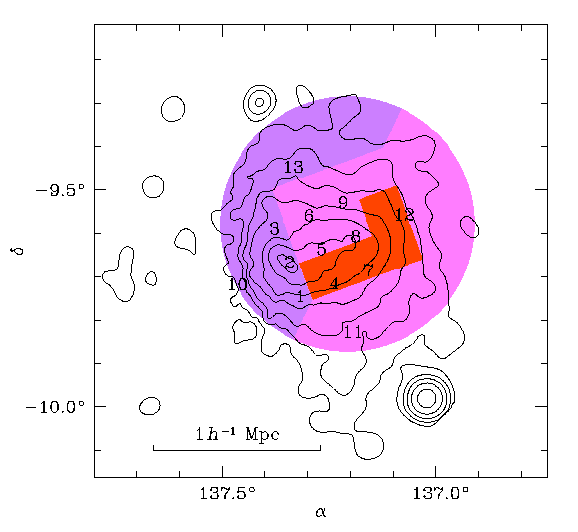
A rich cluster is a complex, multi-component system with hundreds of galaxies, a hot intracluster medium, and dark matter evolving in tightly coupled ways. The properties of clusters are functions both of the processes operating within them and of the underlying cosmology. The determination of cluster properties and their evolution provides fundamental insights into the formation of large scale structure in the universe. Several features make clusters ideal for testing cosmological models. First, clusters are very luminous and are observed both optically and in X-rays to significant distances z ~ 1). Second, since clusters have dynamical timescales which are a significant fraction of the age of the Universe, we can watch them evolve over even modest redshifts. Also since dynamical timescales for clusters are long, the imprint of the initial conditions has not yet been completely erased. Third, since clusters are massive and relatively rare objects, they form only from fairly high peaks in the underlying density field, in the standard scenario. Thus, the cluster mass spectrum is sensitive to the normalization and slope of the power spectrum on cluster scales and is one of the strongest tests of cosmological models. The masses and scales of clusters are such that they comprise a fair sample of the mass of the Universe with representative fractions of the different components. Finally, since cluster formation by gravitational collapse is fairly well understood, comparisons of cluster properties with theoretical predictions for different cosmological models can be used to distinguish among these models.
As described below, with ASCA observations, the important first steps have been taken to understand the structure and distribution of dark matter in present epoch rich clusters, the effects of subcluster mergers on the temperature structure of the gas, the source and likely epoch of the heavy element enrichment of the intracluster medium, and finally how clusters evolve over cosmological times in terms of both their gas temperatures and the heavy element enrichment. As also described, the capabilities of ASCA will allow the cosmological parameters of H_0, q_0, and Omega to be determined in the future as samples of distant clusters are observed.
X-ray imaging spectroscopy, as carried out with ASCA, brings a powerful tool to cluster mass measurements (Bahcall & Sarazin 1977 ApJ 213, L99; Mathews 1978 ApJ 218, 413). The X-ray emitting gas, while itself the major contributor to the luminous mass in clusters, also traces, through the hydrostatic equilibrium equation, the total underlying mass. Since the sound crossing time is short compared to the dynamical timescale for most X-ray luminous clusters, the hydrostatic condition appears applicable over the central few Mpc. While the total mass distributions in cool clusters and groups could be measured with ROSAT (e.g. Ponman & Bertram 1993 Nature 363, 51; David et al. 1994 ApJ 428, 544), the broad energy range of ASCA was required to map the gas temperature in the X-ray luminous, massive clusters. The intracluster medium often shows a central cooler region (e.g. Fabian et al. 1994 PASJ 436, L63), surrounded by an isothermal gas region (e.g. Matsuzawa et al. 1996 Waseda meeting; Markevitch and Vikhlinin 1996 ApJ in press), with lower temperatures sometimes found in the outermost regions (e.g. A2163 - Markevitch et al. 1996 ApJ 456, 437). These accurate determinations of the cluster mass allow the gas mass fraction to be measured and the so-called ``baryon catastrophe'' (the high ratio of gas to total mass) to be examined. ASCA studies are now underway to use observations of a flux-limited sample of clusters to determine the present epoch mass function (Bregman and collaborators).
Although it has long been recognized from both X-ray and optical studies that substructure is common in rich clusters (e.g. Dressler & Shectman 1988 AJ 95, 985; Geller & Beers 1982 PASP 94, 421; Jones & Forman 1992 "Clusters and Superclusters" NATO ASI; Bird 1994 AJ 107, 1637, Mohr et al. 1995 ApJ 447, 8), only with ASCA have we been able to clearly observe the effects of the subcluster mergers on the gas temperature structure within clusters. Following a subcluster merger, shock waves will thermalize and equilibrate the gas within a sound crossing time (~ 2 x 10^9 years), allowing deviations from hydrostatic equilibrium to be observed during this time interval. Recent ASCA observations show non-isothermal temperature structures resulting from mergers. While the map of the X-ray emission from the cluster A754 in Figure 6 shows the asymmetrical nature of this system and hints that a subcluster merger may have occured, the ASCA two-dimensional temperature map still retains the dynamical history of that merger (Henriksen and Markevitch 1996 ApJ in press). The temperature distribution derived from ASCA A754 images is very non-uniform with a significantly hotter ridge found along the cluster axis (Figure 6). The ASCA temperature map for A1367 (Churazov et al. 1995 Wurzburg meeting) shows that the smaller subcluster has the higher temperature, due perhaps to shock heating as the smaller subcluster passed through the larger, cooler subcluster. As these examples show, maps of the cluster temperature provide fundamental information on the dynamical history of these systems which cannot be obtained in any other way.
As described below, with ASCA observations, the important first steps have been taken to understand the structure and distribution of dark matter in present epoch rich clusters, the effects of subcluster mergers on the temperature structure of the gas, the source and likely epoch of the heavy element enrichment of the intracluster medium, and finally how clusters evolve over cosmological times in terms of both their gas temperatures and the heavy element enrichment. As also described, the capabilities of ASCA will allow the cosmological parameters of H_0, q_0, and Omega to be determined in the future as samples of distant clusters are observed.
X-ray imaging spectroscopy, as carried out with ASCA, brings a powerful tool to cluster mass measurements (Bahcall & Sarazin 1977 ApJ 213, L99; Mathews 1978 ApJ 218, 413). The X-ray emitting gas, while itself the major contributor to the luminous mass in clusters, also traces, through the hydrostatic equilibrium equation, the total underlying mass. Since the sound crossing time is short compared to the dynamical timescale for most X-ray luminous clusters, the hydrostatic condition appears applicable over the central few Mpc. While the total mass distributions in cool clusters and groups could be measured with ROSAT (e.g. Ponman & Bertram 1993 Nature 363, 51; David et al. 1994 ApJ 428, 544), the broad energy range of ASCA was required to map the gas temperature in the X-ray luminous, massive clusters. The intracluster medium often shows a central cooler region (e.g. Fabian et al. 1994 PASJ 436, L63), surrounded by an isothermal gas region (e.g. Matsuzawa et al. 1996 Waseda meeting; Markevitch and Vikhlinin 1996 ApJ in press), with lower temperatures sometimes found in the outermost regions (e.g. A2163 - Markevitch et al. 1996 ApJ 456, 437). These accurate determinations of the cluster mass allow the gas mass fraction to be measured and the so-called ``baryon catastrophe'' (the high ratio of gas to total mass) to be examined. ASCA studies are now underway to use observations of a flux-limited sample of clusters to determine the present epoch mass function (Bregman and collaborators).
Although it has long been recognized from both X-ray and optical studies that substructure is common in rich clusters (e.g. Dressler & Shectman 1988 AJ 95, 985; Geller & Beers 1982 PASP 94, 421; Jones & Forman 1992 "Clusters and Superclusters" NATO ASI; Bird 1994 AJ 107, 1637, Mohr et al. 1995 ApJ 447, 8), only with ASCA have we been able to clearly observe the effects of the subcluster mergers on the gas temperature structure within clusters. Following a subcluster merger, shock waves will thermalize and equilibrate the gas within a sound crossing time (~ 2 x 10^9 years), allowing deviations from hydrostatic equilibrium to be observed during this time interval. Recent ASCA observations show non-isothermal temperature structures resulting from mergers. While the map of the X-ray emission from the cluster A754 in Figure 6 shows the asymmetrical nature of this system and hints that a subcluster merger may have occured, the ASCA two-dimensional temperature map still retains the dynamical history of that merger (Henriksen and Markevitch 1996 ApJ in press). The temperature distribution derived from ASCA A754 images is very non-uniform with a significantly hotter ridge found along the cluster axis (Figure 6). The ASCA temperature map for A1367 (Churazov et al. 1995 Wurzburg meeting) shows that the smaller subcluster has the higher temperature, due perhaps to shock heating as the smaller subcluster passed through the larger, cooler subcluster. As these examples show, maps of the cluster temperature provide fundamental information on the dynamical history of these systems which cannot be obtained in any other way.
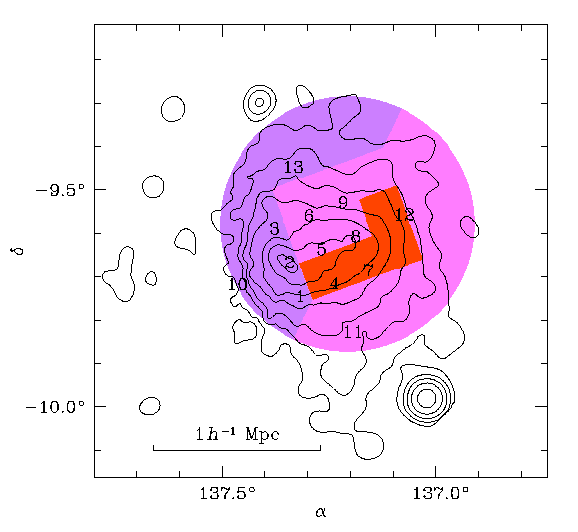
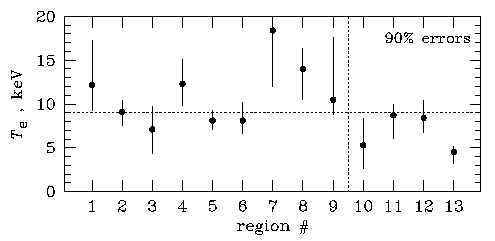
Figure 6. X-ray intensity and temperature maps for Abell 754 from Henriksen & Markevitch (1996 ApJ in press).
While much of the hot ICM is primordial material, this gas has been enriched in heavy elements. The ASCA observations have shown that, in individual subclusters, such as those noted above, and for all measured rich clusters, the heavy element abundances are remarkably constant at ~1/3 the solar abundance (Ohashi 1995 Wurzburg meeting). The constant abundance ratios, as well as the constant mass to light ratios, suggest that the ratio of galaxies' mass to primordial mass is also constant and that the observed increase in the ratio of gas mass to galaxy mass with cluster scale results from mass loss in the less massive systems (David 1996). The source of the heavy element enrichment can be determined from the ASCA spectra. Measurements of Si and S abundances compared to that of iron from ASCA observations demonstrate that Type II supernovae at an early epoch are predominantly responsible for the heavy element enrichment in clusters (e.g. Mushotzky et al. 1996 ApJ in press, Hatsukade et al. 1996 Waseda meeting). In some cD clusters, ASCA observations suggest that higher abundances occur around the cD galaxy perhaps due to perhaps to the confinement of heavily enriched material generated and ejected by the central galaxy (Fukasawa et al. 1996).
Studies are currently underway to determine H_0 from ASCA and ROSAT X-ray observations. Combining the measured values of the cluster gas temperature and electron number density with radio observations of the Sunyaev-Zel'dovich effect (the decrement in the radio flux which results as cosmic microwave background photons pass through the hot plasma of a cluster and are shifted to higher energy) (e.g. Hughes and Birkinshaw 1996 ApJ submitted) will yield direct measures of H_0 to the clusters being studied.
ASCA observations of distant (z ~ 0.3), X-ray luminous clusters selected from optical catalogs, as well as from the Einstein Medium Sensitivity Survey and the ROSAT All Sky Survey, indicate that the abundance of heavy elements is fully consistent with that found at low redshifts (Bautz et al. 1994 PASJ 46, L131; Donahue 1996 ApJ in press; Henry 1996 in preparation; Tsuru et al. 1996 Waseda meeting; Jones et al. 1996 in preparation), thereby supporting an early epoch for the enrichment. ASCA observations of one distant cluster at z=1.0, found in the search for a QSO lens, detected the redshifted iron line at 3.35 keV with an abundance of 0.3 solar (Hattori et al. 1995 Wurzburg meeting). The high redshift clusters show a luminosity-temperature relation similar to that observed in present epoch clusters (Mushotzky 1995 Wurzburg meeting; Henry 1996 in preparation; Kumada et al. 1996 Waseda meeting).
In the future as larger, complete samples of clusters, particularly those at high redshift become available, it will be possible to place constraints on cosmological models which predict the cluster mass function, gas mass fraction, ICM temperatures, and heavy element enrichment in the gas. Distant clusters observed by ASCA will provide an earlier ``snapshot'' of cluster evolution. Such samples have been observed or are planned for ASCA observations. The cluster mass function, which can be derived from these observations, is directly traceable to the underlying cosmology and, unlike some other cluster properties, is not susceptible to modification by non-gravitational processes. The mass function provides the normalization and slope for the mass fluctuation spectrum on scales from 10^13 to 10^15 Solar masses. Currently, with only the present epoch ``snapshot,'' our confidence in the comparison of observation with theory remains uncertain. Critical information for distinguishing among models is derived by measuring X-ray cluster properties as a function of redshift. Not only must a model predict the present epoch cluster properties, but it must also describe clusters at earlier epochs.
The scant available observational data already exclude some models. For example, standard COBE normalized CDM models predict too many luminous clusters by a factor of five (Bryan et al. 1994 ApJ 437, L5). ASCA images can determine the present epoch mass function by directly measuring the masses for a cluster sample similar to that of Henry and Arnaud. From ASCA observations, mass functions at high redshift can be compared with the present epoch mass function. The sensitivity and importance of measuring the mass function at different epochs can be seen by comparing the comoving cluster number densities for different cosmologies. For example, Viana and Liddle (1995 preprint) show that at z = 0.8, the number of hot clusters (around 7 keV) for three popular cosmological models - standard biased CDM, a flat open model with Omega = 0.3 and Lambda = 0.7, and an open CDM model with Omega = 0.3 - are in the ratio of 1:60:300 (note, this ratio is converted from volume density to the observable, number per sq. degree). Thus, comparisons of cluster mass functions from different redshifts provide stringent tests of cosmological models.
The mean temperatures of cluster samples at different epochs also is sensitive to the assumed cosmological model (Cen & Ostriker 1994 ApJ 431, 451; Jing & Fang 1994 ApJ 432, 438). For example, for self-similar hierarchical models with a steep spectrum of primordial fluctuations to explain the reported X-ray luminosity evolution, clusters at z = 0.3 should have higher gas densities than at present, be two times cooler, be two times more compact and at low energies, be more X-ray luminous. These are large effects that can be tested with future ASCA observations.
Finally, since the effective area of the ASCA telescopes at 6 keV exceeds that of AXAF, it may be efficient in terms of observing time to measure the iron abundance for clusters with ASCA while obtaining high resolution images with AXAF.
Last modified: Tuesday, 26-Jun-2001 14:22:33 EDT
If you have any questions concerning ASCA, visit our Feedback form.